Polar amplification

Polar amplification is the phenomenon that any change in the net radiation balance (for example greenhouse intensification) tends to produce a larger change in temperature near the poles than in the planetary average.[1] This is commonly referred to as the ratio of polar warming to tropical warming. On a planet with an atmosphere that can restrict emission of longwave radiation to space (a greenhouse effect), surface temperatures will be warmer than a simple planetary equilibrium temperature calculation would predict. Where the atmosphere or an extensive ocean is able to transport heat polewards, the poles will be warmer and equatorial regions cooler than their local net radiation balances would predict.[2] The poles will experience the most cooling when the global-mean temperature is lower relative to a reference climate; alternatively, the poles will experience the greatest warming when the global-mean temperature is higher.[1]
In the extreme, the planet Venus is thought to have experienced a very large increase in greenhouse effect over its lifetime,[3] so much so that its poles have warmed sufficiently to render its surface temperature effectively isothermal (no difference between poles and equator).[4][5] On Earth, water vapor and trace gasses provide a lesser greenhouse effect, and the atmosphere and extensive oceans provide efficient poleward heat transport. Both palaeoclimate changes and recent global warming changes have exhibited strong polar amplification, as described below.
Arctic amplification is polar amplification of the Earth's North Pole only; Antarctic amplification is that of the South Pole.
History
An observation-based study related to Arctic amplification was published in 1969 by Mikhail Budyko,[6] and the study conclusion has been summarized as "Sea ice loss affects Arctic temperatures through the surface albedo feedback."[7][8] The same year, a similar model was published by William D. Sellers.[9] Both studies attracted significant attention since they hinted at the possibility for a runaway positive feedback within the global climate system.[10] In 1975, Manabe and Wetherald published the first somewhat plausible general circulation model that looked at the effects of an increase of greenhouse gas. Although confined to less than one-third of the globe, with a "swamp" ocean and only land surface at high latitudes, it showed an Arctic warming faster than the tropics (as have all subsequent models).[11]
Amplification
Amplifying mechanisms
Feedbacks associated with sea ice and snow cover are widely cited as one of the principal causes of terrestrial polar amplification.[12][13][14] These feedbacks are particularly noted in local polar amplification,[15] although recent work has shown that the lapse rate feedback is likely equally important to the ice-albedo feedback for Arctic amplification.[16] Supporting this idea, large-scale amplification is also observed in model worlds with no ice or snow.[17] It appears to arise both from a (possibly transient) intensification of poleward heat transport and more directly from changes in the local net radiation balance.[17] Local radiation balance is crucial because an overall decrease in outgoing longwave radiation will produce a larger relative increase in net radiation near the poles than near the equator.[16] Thus, between the lapse rate feedback and changes in the local radiation balance, much of polar amplification can be attributed to changes in outgoing longwave radiation.[15][18] This is especially true for the Arctic, whereas the elevated terrain in Antarctica limits the influence of the lapse rate feedback.[16][19]
Some examples of climate system feedbacks thought to contribute to recent polar amplification include the reduction of snow cover and sea ice, changes in atmospheric and ocean circulation, the presence of anthropogenic soot in the Arctic environment, and increases in cloud cover and water vapor.[13] CO2 forcing has also been attributed to polar amplification.[20] Most studies connect sea ice changes to polar amplification.[13] Both ice extent and thickness impact polar amplification. Climate models with smaller baseline sea ice extent and thinner sea ice coverage exhibit stronger polar amplification.[21] Some models of modern climate exhibit Arctic amplification without changes in snow and ice cover.[22]
The individual processes contributing to polar warming are critical to understanding climate sensitivity.[23] Polar warming also affects many ecosystems, including marine and terrestrial ecosystems, climate systems, and human populations.[20] Polar amplification is largely driven by local polar processes with hardly any remote forcing, whereas polar warming is regulated by tropical and midlatitude forcing.[24] These impacts of polar amplification have led to continuous research in the face of global warming.
Ocean circulation
It has been estimated that 70% of global wind energy is transferred to the ocean and takes place within the Antarctic Circumpolar Current (ACC).[25] Eventually, upwelling due to wind-stress transports cold Antarctic waters through the Atlantic surface current, while warming them over the equator, and into the Arctic environment. This is especially noticed in high latitudes.[21] Thus, warming in the Arctic depends on the efficiency of the global ocean transport and plays a role in the polar see-saw effect.[25]
Decreased oxygen and low-pH during La Niña are processes that correlate with decreased primary production and a more pronounced poleward flow of ocean currents.[26] It has been proposed that the mechanism of increased Arctic surface air temperature anomalies during La Niña periods of ENSO may be attributed to the Tropically Excited Arctic Warming Mechanism (TEAM), when Rossby waves propagate more poleward, leading to wave dynamics and an increase in downward infrared radiation.[1][27]
Amplification factor
Polar amplification is quantified in terms of a polar amplification factor, generally defined as the ratio of some change in a polar temperature to a corresponding change in a broader average temperature:
- ,
where is a change in polar temperature and is, for example, a corresponding change in a global mean temperature.
Common implementations[28][29] define the temperature changes directly as the anomalies in surface air temperature relative to a recent reference interval (typically 30 years). Others have used the ratio of the variances of surface air temperature over an extended interval.[30]
Amplification phase
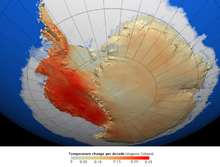
It is observed that Arctic and Antarctic warming commonly proceed out of phase because of orbital forcing, resulting in the so-called polar see-saw effect.[31]
Paleoclimate polar amplification
The glacial / interglacial cycles of the Pleistocene provide extensive palaeoclimate evidence of polar amplification, both from the Arctic and the Antarctic.[29] In particular, the temperature rise since the last glacial maximum 20,000 years ago provides a clear picture. Proxy temperature records from the Arctic (Greenland) and from the Antarctic indicate polar amplification factors on the order of 2.0.[29]
Recent Arctic amplification

Suggested mechanisms leading to the observed Arctic amplification include Arctic sea ice decline (open water reflects less sunlight than sea ice), atmospheric heat transport from the equator to the Arctic,[33] and the lapse rate feedback.[16]
The Arctic was historically described as warming twice as fast as the global average,[34] but this estimate was based on older observations which missed the more recent acceleration. By 2021, enough data was available to show that the Arctic had warmed three times as fast as the globe - 3.1°C between 1971 and 2019, as opposed to the global warming of 1°C over the same period.[35] Moreover, this estimate defines the Arctic as everything above 60th parallel north, or a full third of the Northern Hemisphere: in 2021–2022, it was found that since 1979, the warming within the Arctic Circle itself (above the 66th parallel) has been nearly four times faster than the global average.[36][37] Within the Arctic Circle itself, even greater Arctic amplification occurs in the Barents Sea area, with hotspots around West Spitsbergen Current: weather stations located on its path record decadal warming up to seven times faster than the global average.[38][39] This has fuelled concerns that unlike the rest of the Arctic sea ice, ice cover in the Barents Sea may permanently disappear even around 1.5 degrees of global warming.[40][41]
The acceleration of Arctic amplification has not been linear: a 2022 analysis found that it occurred in two sharp steps, with the former around 1986, and the latter after 2000.[42] The first acceleration is attributed to the increase in anthropogenic radiative forcing in the region, which is in turn likely connected to the reductions in stratospheric sulfur aerosols pollution in Europe in the 1980s in order to combat acid rain. Since sulphate aerosols have a cooling effect, their absence is likely to have increased Arctic temperatures by up to 0.5 degrees Celsius.[43][44] The second acceleration has no known cause,[35] which is why it did not show up in any climate models. It is likely to be an example of multi-decadal natural variability, like the suggested link between Arctic temperatures and Atlantic Multi-decadal Oscillation (AMO),[45] in which case it can be expected to reverse in the future. However, even the first increase in Arctic amplification was only accurately simulated by a fraction of the current CMIP6 models.[42]
Possible impacts on mid-latitude weather
Since the early 2000s, climate models have consistently identified that global warming will gradually push jet streams poleward. In 2008, this was confirmed by observational evidence, which proved that from 1979 to 2001, the northern jet stream moved northward at an average rate of 2.01 kilometres (1.25 mi) per year, with a similar trend in the southern hemisphere jet stream.[46][47] Climate scientists have hypothesized that the jet stream will also gradually weaken as a result of global warming. Trends such as Arctic sea ice decline, reduced snow cover, evapotranspiration patterns, and other weather anomalies have caused the Arctic to heat up faster than other parts of the globe, in what is known as the Arctic amplification. In 2021–2022, it was found that since 1979, the warming within the Arctic Circle has been nearly four times faster than the global average,[48][49] and some hotspots in the Barents Sea area warmed up to seven times faster than the global average.[50][51] While the Arctic remains one of the coldest places on Earth today, the temperature gradient between it and the warmer parts of the globe will continue to diminish with every decade of global warming as the result of this amplification. If this gradient has a strong influence on the jet stream, then it will eventually become weaker and more variable in its course, which would allow more cold air from the polar vortex to leak mid-latitudes and slow the progression of Rossby waves, leading to more persistent and more extreme weather.[52]
The hypothesis above is closely associated with Jennifer Francis, who had first proposed it in a 2012 paper co-authored by Stephen J. Vavrus.[52] While some paleoclimate reconstructions have suggested that the polar vortex becomes more variable and causes more unstable weather during periods of warming back in 1997,[53] this was contradicted by climate modelling, with PMIP2 simulations finding in 2010 that the Arctic Oscillation (AO) was much weaker and more negative during the Last Glacial Maximum, and suggesting that warmer periods have stronger positive phase AO, and thus less frequent leaks of the polar vortex air.[54] However, a 2012 review in the Journal of the Atmospheric Sciences noted that "there [has been] a significant change in the vortex mean state over the twenty-first century, resulting in a weaker, more disturbed vortex.",[55] which contradicted the modelling results but fit the Francis-Vavrus hypothesis. Additionally, a 2013 study noted that the then-current CMIP5 tended to strongly underestimate winter blocking trends,[56] and other 2012 research had suggested a connection between declining Arctic sea ice and heavy snowfall during midlatitude winters.[57]
In 2013, further research from Francis connected reductions in the Arctic sea ice to extreme summer weather in the northern mid-latitudes,[58] while other research from that year identified potential linkages between Arctic sea ice trends and more extreme rainfall in the European summer.[59] At the time, it was also suggested that this connection between Arctic amplification and jet stream patterns was involved in the formation of Hurricane Sandy[60] and played a role in the early 2014 North American cold wave.[61][62] In 2015, Francis' next study concluded that highly amplified jet-stream patterns are occurring more frequently in the past two decades. Hence, continued heat-trapping emissions favour increased formation of extreme events caused by prolonged weather conditions.[63]
Studies published in 2017 and 2018 identified stalling patterns of Rossby waves in the northern hemisphere jet stream as the culprit behind other almost stationary extreme weather events, such as the 2018 European heatwave, the 2003 European heat wave, 2010 Russian heat wave or the 2010 Pakistan floods, and suggested that these patterns were all connected to Arctic amplification.[64][65] Further work from Francis and Vavrus that year suggested that amplified Arctic warming is observed as stronger in lower atmospheric areas because the expanding process of warmer air increases pressure levels which decreases poleward geopotential height gradients. As these gradients are the reason that cause west to east winds through the thermal wind relationship, declining speeds are usually found south of the areas with geopotential increases.[66] In 2017, Francis explained her findings to the Scientific American: "A lot more water vapor is being transported northward by big swings in the jet stream. That's important because water vapor is a greenhouse gas just like carbon dioxide and methane. It traps heat in the atmosphere. That vapor also condenses as droplets we know as clouds, which themselves trap more heat. The vapor is a big part of the amplification story—a big reason the Arctic is warming faster than anywhere else."[67]
In a 2017 study conducted by climatologist Judah Cohen and several of his research associates, Cohen wrote that "[the] shift in polar vortex states can account for most of the recent winter cooling trends over Eurasian midlatitudes".[68] A 2018 paper from Vavrus and others linked Arctic amplification to more persistent hot-dry extremes during the midlatitude summers, as well as the midlatitude winter continental cooling.[69] Another 2017 paper estimated that when the Arctic experiences anomalous warming, primary production in North America goes down by between 1% and 4% on average, with some states suffering up to 20% losses.[70] A 2021 study found that a stratospheric polar vortex disruption is linked with extreme cold winter weather across parts of Asia and North America, including the February 2021 North American cold wave.[71][72] Another 2021 study identified a connection between the Arctic sea ice loss and the increased size of wildfires in the Western United States.[73]
However, because the specific observations are considered short-term observations, there is considerable uncertainty in the conclusions. Climatology observations require several decades to definitively distinguish various forms of natural variability from climate trends.[74] This point was stressed by reviews in 2013[75] and in 2017.[76] A study in 2014 concluded that Arctic amplification significantly decreased cold-season temperature variability over the northern hemisphere in recent decades. Cold Arctic air intrudes into the warmer lower latitudes more rapidly today during autumn and winter, a trend projected to continue in the future except during summer, thus calling into question whether winters will bring more cold extremes.[77] A 2019 analysis of a data set collected from 35 182 weather stations worldwide, including 9116 whose records go beyond 50 years, found a sharp decrease in northern midlatitude cold waves since the 1980s.[78]
Moreover, a range of long-term observational data collected during the 2010s and published in 2020 suggests that the intensification of Arctic amplification since the early 2010s was not linked to significant changes on mid-latitude atmospheric patterns.[79][80] State-of-the-art modelling research of PAMIP (Polar Amplification Model Intercomparison Project) improved upon the 2010 findings of PMIP2; it found that sea ice decline would weaken the jet stream and increase the probability of atmospheric blocking, but the connection was very minor, and typically insignificant next to interannual variability.[81][82] In 2022, a follow-up study found that while the PAMIP average had likely underestimated the weakening caused by sea ice decline by 1.2 to 3 times, even the corrected connection still amounts to only 10% of the jet stream's natural variability.[83]
Additionally, a 2021 study found that while jet streams had indeed slowly moved polewards since 1960 as was predicted by models, they did not weaken, in spite of a small increase in waviness.[84] A 2022 re-analysis of the aircraft observational data collected over 2002–2020 suggested that the North Atlantic jet stream had actually strengthened.[85] Finally, a 2021 study was able to reconstruct jet stream patterns over the past 1,250 years based on Greenland ice cores, and found that all of the recently observed changes remain within range of natural variability: the earliest likely time of divergence is in 2060, under the Representative Concentration Pathway 8.5 which implies continually accelerating greenhouse gas emissions.[86]See also
- Arctic dipole anomaly
- Arctic oscillation
- Climate of the Arctic
- Polar vortex
- Sudden stratospheric warming
References
- ^ a b c Lee, Sukyoung (January 2014). "A theory for polar amplification from a general circulation perspective" (PDF). Asia-Pacific Journal of the Atmospheric Sciences. 50 (1): 31–43. Bibcode:2014APJAS..50...31L. doi:10.1007/s13143-014-0024-7. S2CID 20639425.
- ^ Pierrehumbert, R. T. (2010). Principles of Planetary Climate. Cambridge University Press. ISBN 978-0-521-86556-2.
- ^ Kasting, J. F. (1988). "Runaway and moist greenhouse atmospheres and the evolution of Earth and Venus". Icarus. 74 (3): 472–94. Bibcode:1988Icar...74..472K. doi:10.1016/0019-1035(88)90116-9. PMID 11538226.
- ^ Williams, David R. (15 April 2005). "Venus Fact Sheet". NASA. Retrieved 2007-10-12.
- ^ Lorenz, Ralph D.; Lunine, Jonathan I.; Withers, Paul G.; McKay, Christopher P. (2001). "Titan, Mars and Earth: Entropy Production by Latitudinal Heat Transport" (PDF). Ames Research Center, University of Arizona Lunar and Planetary Laboratory. Retrieved 2007-08-21.
- ^ Budyko, M.I. (1969). "The effect of solar radiation variations on the climate of the Earth". Tellus. 21 (5): 611–9. Bibcode:1969Tell...21..611B. doi:10.3402/tellusa.v21i5.10109. S2CID 21745322.
- ^ Cvijanovic, Ivana; Caldeira, Ken (2015). "Atmospheric impacts of sea ice decline in CO2 induced global warming" (PDF). Climate Dynamics. 44 (5–6): 1173–86. Bibcode:2015ClDy...44.1173C. doi:10.1007/s00382-015-2489-1. S2CID 106405448.
- ^ "Ice in Action: Sea ice at the North Pole has something to say about climate change". YaleScientific. 2016.
- ^ Sellers, William D. (1969). "A Global Climatic Model Based on the Energy Balance of the Earth-Atmosphere System". Journal of Applied Meteorology. 8 (3): 392–400. Bibcode:1969JApMe...8..392S. doi:10.1175/1520-0450(1969)008<0392:AGCMBO>2.0.CO;2.
- ^ Oldfield, Jonathan D. (2016). "Mikhail Budyko's (1920–2001) contributions to Global Climate Science: from heat balances to climate change and global ecology". Advanced Review. 7 (5): 682–692. Bibcode:2016WIRCC...7..682O. doi:10.1002/wcc.412.
- ^ Manabe, Syukoro; Wetherald, Richard T. (1975). "The Effects of Doubling the CO2 Concentration on the Climate of a General Circulation Model". Journal of the Atmospheric Sciences. 32 (1): 3–15. Bibcode:1975JAtS...32....3M. doi:10.1175/1520-0469(1975)032<0003:TEODTC>2.0.CO;2.
- ^ Hansen J, Sato M, Ruedy R (1997). "Radiative forcing and climate response". Journal of Geophysical Research: Atmospheres. 102 (D6): 6831–64. Bibcode:1997JGR...102.6831H. doi:10.1029/96jd03436.
- ^ a b c "IPCC AR5 – Near-term Climate Change: Projections and Predictability (Chapter 11 / page 983 )" (PDF). 2013.
- ^ Pistone, Kristina; Eisenman, Ian; Ramanathan, Veerabhadran (2019). "Radiative Heating of an Ice-Free Arctic Ocean". Geophysical Research Letters. 46 (13): 7474–7480. Bibcode:2019GeoRL..46.7474P. doi:10.1029/2019GL082914. S2CID 197572148.
- ^ a b Bekryaev, Roman V.; Polyakov, Igor V.; Alexeev, Vladimir A. (2010-07-15). "Role of Polar Amplification in Long-Term Surface Air Temperature Variations and Modern Arctic Warming". Journal of Climate. 23 (14): 3888–3906. Bibcode:2010JCli...23.3888B. doi:10.1175/2010JCLI3297.1. ISSN 0894-8755.
- ^ a b c d Goosse, Hugues; Kay, Jennifer E.; Armour, Kyle C.; Bodas-Salcedo, Alejandro; Chepfer, Helene; Docquier, David; Jonko, Alexandra; Kushner, Paul J.; Lecomte, Olivier; Massonnet, François; Park, Hyo-Seok; Pithan, Felix; Svensson, Gunilla; Vancoppenolle, Martin (December 2018). "Quantifying climate feedbacks in polar regions". Nature Communications. 9 (1): 1919. Bibcode:2018NatCo...9.1919G. doi:10.1038/s41467-018-04173-0. PMC 5953926. PMID 29765038.
- ^ a b Alexeev VA, Langen PL, Bates JR (2005). "Polar amplification of surface warming on an aquaplanet in "ghost forcing" experiments without sea ice feedbacks". Climate Dynamics. 24 (7–8): 655–666. Bibcode:2005ClDy...24..655A. doi:10.1007/s00382-005-0018-3. S2CID 129600712.
- ^ Payne, Ashley E.; Jansen, Malte F.; Cronin, Timothy W. (2015). "Conceptual model analysis of the influence of temperature feedbacks on polar amplification". Geophysical Research Letters. 42 (21): 9561–9570. Bibcode:2015GeoRL..42.9561P. doi:10.1002/2015GL065889. ISSN 1944-8007.
- ^ Hahn, L. C.; Armour, K. C.; Battisti, D. S.; Donohoe, A.; Pauling, A. G.; Bitz, C. M. (28 August 2020). "Antarctic Elevation Drives Hemispheric Asymmetry in Polar Lapse Rate Climatology and Feedback". Geophysical Research Letters. 47 (16). Bibcode:2020GeoRL..4788965H. doi:10.1029/2020GL088965. S2CID 222009674.
- ^ a b Stuecker, Malte F.; Bitz, Cecilia M.; Armour, Kyle C.; Proistosescu, Cristian; Kang, Sarah M.; Xie, Shang Ping; Kim, Doyeon; McGregor, Shayne; Zhang, Wenjun; Zhao, Sen; Cai, Wenju (December 2018). "Polar amplification dominated by local forcing and feedbacks". Nature Climate Change. 8 (12): 1076–1081. Bibcode:2018NatCC...8.1076S. doi:10.1038/s41558-018-0339-y. ISSN 1758-6798. S2CID 92195853.
- ^ a b Holland, M. M.; Bitz, C. M. (2003-09-01). "Polar amplification of climate change in coupled models". Climate Dynamics. 21 (3): 221–232. Bibcode:2003ClDy...21..221H. doi:10.1007/s00382-003-0332-6. ISSN 1432-0894. S2CID 17003665.
- ^ Pithan, Felix; Mauritsen, Thorsten (February 2, 2014). "Arctic amplification dominated by temperature feedbacks in contemporary climate models". Nature Geoscience. 7 (3): 181–4. Bibcode:2014NatGe...7..181P. doi:10.1038/ngeo2071. S2CID 140616811.
- ^ Taylor, Patrick C.; Cai, Ming; Hu, Aixue; Meehl, Jerry; Washington, Warren; Zhang, Guang J. (2013-09-09). "A Decomposition of Feedback Contributions to Polar Warming Amplification". Journal of Climate. 26 (18). American Meteorological Society: 7023–7043. Bibcode:2013JCli...26.7023T. doi:10.1175/jcli-d-12-00696.1. ISSN 0894-8755.
- ^ Stuecker, Malte F.; Bitz, Cecilia M.; Armour, Kyle C.; Proistosescu, Cristian; Kang, Sarah M.; Xie, Shang-Ping; Kim, Doyeon; McGregor, Shayne; Zhang, Wenjun; Zhao, Sen; Cai, Wenju; Dong, Yue; Jin, Fei-Fei (December 2018). "Polar amplification dominated by local forcing and feedbacks". Nature Climate Change. 8 (12): 1076–1081. Bibcode:2018NatCC...8.1076S. doi:10.1038/s41558-018-0339-y. ISSN 1758-6798. S2CID 92195853.
- ^ a b Petr Chylek; Chris K. Folland; Glen Lesins; Manvendra K. Dubey (February 3, 2010). "Twentieth century bipolar seesaw of the Arctic and Antarctic surface air temperatures" (PDF). Geophysical Research Letters. 12 (8): 4015–22. Bibcode:2010GeoRL..37.8703C. doi:10.1029/2010GL042793. S2CID 18491097. Archived from the original (PDF) on February 20, 2014. Retrieved May 1, 2014.
- ^ Sung Hyun Nam; Hey-Jin Kim; Uwe Send (November 23, 2011). "Amplification of hypoxic and acidic events by La Niña conditions on the continental shelf off California". Geophysical Research Letters. 83 (22): L22602. Bibcode:2011GeoRL..3822602N. doi:10.1029/2011GL049549. S2CID 55150106.
- ^ Sukyoung Lee (June 2012). "Testing of the Tropically Excited Arctic Warming Mechanism (TEAM) with Traditional El Niño and La Niña". Journal of Climate. 25 (12): 4015–22. Bibcode:2012JCli...25.4015L. doi:10.1175/JCLI-D-12-00055.1. S2CID 91176052.
- ^ Masson-Delmotte, V.; M. Kageyama; P. Braconnot; S. Charbit; G. Krinner; C. Ritz; E. Guilyardi; et al. (2006). "Past and future polar amplification of climate change: climate model intercomparisons and ice-core constraints". Climate Dynamics. 26 (5): 513–529. Bibcode:2006ClDy...26..513M. doi:10.1007/s00382-005-0081-9. S2CID 2370836.
- ^ a b c James Hansen; Makiko Sato; Gary Russell; Pushker Kharecha (September 2013). "Climate sensitivity, sea level and atmospheric carbon dioxide". Philosophical Transactions of the Royal Society A. 371 (2001). arXiv:1211.4846. Bibcode:2013RSPTA.37120294H. doi:10.1098/rsta.2012.0294. PMC 3785813. PMID 24043864.
- ^ Kobashi, T.; Shindell, D. T.; Kodera, K.; Box, J. E.; Nakaegawa, T.; Kawamura, K. (2013). "On the origin of multidecadal to centennial Greenland temperature anomalies over the past 800 yr". Climate of the Past. 9 (2): 583–596. Bibcode:2013CliPa...9..583K. doi:10.5194/cp-9-583-2013. hdl:2060/20150002680.
- ^ Kyoung-nam Jo; Kyung Sik Woo; Sangheon Yi; Dong Yoon Yang; Hyoun Soo Lim; Yongjin Wang; Hai Cheng; R. Lawrence Edwards (March 30, 2014). "Mid-latitude interhemispheric hydrologic seesaw over the past 550,000 years". Nature. 508 (7496): 378–382. Bibcode:2014Natur.508..378J. doi:10.1038/nature13076. PMID 24695222. S2CID 2096406.
- ^ "Thermodynamics: Albedo". NSIDC.
- ^ "Arctic amplification". NASA. 2013.
- ^ "Polar Vortex: How the Jet Stream and Climate Change Bring on Cold Snaps". InsideClimate News. 2018-02-02. Retrieved 2018-11-24.
- ^ a b "Arctic warming three times faster than the planet, report warns". Phys.org. 2021-05-20. Retrieved 6 October 2022.
- ^ Rantanen, Mika; Karpechko, Alexey Yu; Lipponen, Antti; Nordling, Kalle; Hyvärinen, Otto; Ruosteenoja, Kimmo; Vihma, Timo; Laaksonen, Ari (11 August 2022). "The Arctic has warmed nearly four times faster than the globe since 1979". Communications Earth & Environment. 3 (1): 168. Bibcode:2022ComEE...3..168R. doi:10.1038/s43247-022-00498-3. hdl:11250/3115996. ISSN 2662-4435. S2CID 251498876.
- ^ "The Arctic is warming four times faster than the rest of the world". 2021-12-14. Retrieved 6 October 2022.
- ^ Isaksen, Ketil; Nordli, Øyvind; et al. (15 June 2022). "Exceptional warming over the Barents area". Scientific Reports. 12 (1): 9371. Bibcode:2022NatSR..12.9371I. doi:10.1038/s41598-022-13568-5. PMC 9200822. PMID 35705593. S2CID 249710630.
- ^ Damian Carrington (2022-06-15). "New data reveals extraordinary global heating in the Arctic". The Guardian. Retrieved 7 October 2022.
- ^ Armstrong McKay, David; Abrams, Jesse; Winkelmann, Ricarda; Sakschewski, Boris; Loriani, Sina; Fetzer, Ingo; Cornell, Sarah; Rockström, Johan; Staal, Arie; Lenton, Timothy (9 September 2022). "Exceeding 1.5°C global warming could trigger multiple climate tipping points". Science. 377 (6611): eabn7950. doi:10.1126/science.abn7950. hdl:10871/131584. ISSN 0036-8075. PMID 36074831. S2CID 252161375.
- ^ Armstrong McKay, David (9 September 2022). "Exceeding 1.5°C global warming could trigger multiple climate tipping points – paper explainer". climatetippingpoints.info. Retrieved 2 October 2022.
- ^ a b Chylek, Petr; Folland, Chris; Klett, James D.; Wang, Muyin; Hengartner, Nick; Lesins, Glen; Dubey, Manvendra K. (25 June 2022). "Annual Mean Arctic Amplification 1970–2020: Observed and Simulated by CMIP6 Climate Models". Geophysical Research Letters. 49 (13). Bibcode:2022GeoRL..4999371C. doi:10.1029/2022GL099371. S2CID 250097858.
- ^ Acosta Navarro, J.C.; Varma, V.; Riipinen, I.; Seland, Ø.; Kirkevåg, A.; Struthers, H.; Iversen, T.; Hansson, H.-C.; Ekman, A. M. L. (14 March 2016). "Amplification of Arctic warming by past air pollution reductions in Europe". Nature Geoscience. 9 (4): 277–281. Bibcode:2016NatGe...9..277A. doi:10.1038/ngeo2673.
- ^ Harvey, C. (14 March 2016). "How cleaner air could actually make global warming worse". Washington Post.
- ^ Chylek, Petr; Folland, Chris K.; Lesins, Glen; Dubey, Manvendra K.; Wang, Muyin (16 July 2009). "Arctic air temperature change amplification and the Atlantic Multidecadal Oscillation". Geophysical Research Letters. 36 (14): L14801. Bibcode:2009GeoRL..3614801C. CiteSeerX 10.1.1.178.6926. doi:10.1029/2009GL038777. S2CID 14013240.
- ^ Archer, Cristina L.; Caldeira, Ken (18 April 2008). "Historical trends in the jet streams". Geophysical Research Letters. 35 (8). Bibcode:2008GeoRL..35.8803A. doi:10.1029/2008GL033614. S2CID 59377392.
- ^ "Jet stream found to be permanently drifting north". Associated Press. 2008-04-18. Archived from the original on 17 August 2016. Retrieved 7 October 2022.
- ^ Rantanen, Mika; Karpechko, Alexey Yu; Lipponen, Antti; Nordling, Kalle; Hyvärinen, Otto; Ruosteenoja, Kimmo; Vihma, Timo; Laaksonen, Ari (11 August 2022). "The Arctic has warmed nearly four times faster than the globe since 1979". Communications Earth & Environment. 3 (1): 168. Bibcode:2022ComEE...3..168R. doi:10.1038/s43247-022-00498-3. hdl:11250/3115996. ISSN 2662-4435. S2CID 251498876.
- ^ "The Arctic is warming four times faster than the rest of the world". Science Magazine. 2021-12-14. Archived from the original on 8 November 2023. Retrieved 6 October 2022.
- ^ Isaksen, Ketil; Nordli, Øyvind; et al. (15 June 2022). "Exceptional warming over the Barents area". Scientific Reports. 12 (1): 9371. Bibcode:2022NatSR..12.9371I. doi:10.1038/s41598-022-13568-5. PMC 9200822. PMID 35705593.
- ^ Damian Carrington (2022-06-15). "New data reveals extraordinary global heating in the Arctic". The Guardian. Archived from the original on 1 October 2023. Retrieved 7 October 2022.
- ^ a b Francis, Jennifer A.; Vavrus, Stephen J. (2012). "Evidence linking Arctic amplification to extreme weather in mid-latitudes". Geophysical Research Letters. 39 (6): L06801. Bibcode:2012GeoRL..39.6801F. CiteSeerX 10.1.1.419.8599. doi:10.1029/2012GL051000. S2CID 15383119.
- ^ Zielinski, G.; Mershon, G. (1997). "Paleoenvironmental implications of the insoluble microparticle record in the GISP2 (Greenland) ice core during the rapidly changing climate of the Pleistocene-Holocene transition". Bulletin of the Geological Society of America. 109 (5): 547–559. Bibcode:1997GSAB..109..547Z. doi:10.1130/0016-7606(1997)109<0547:piotim>2.3.co;2.
- ^ Lue, J.-M.; Kim, S.-J.; Abe-Ouchi, A.; Yu, Y.; Ohgaito, R. (2010). "Arctic Oscillation during the Mid-Holocene and Last Glacial Maximum from PMIP2 Coupled Model Simulations". Journal of Climate. 23 (14): 3792–3813. Bibcode:2010JCli...23.3792L. doi:10.1175/2010JCLI3331.1 (inactive 2 December 2024). S2CID 129156297.
{{cite journal}}
: CS1 maint: DOI inactive as of December 2024 (link) - ^ Mitchell, Daniel M.; Osprey, Scott M.; Gray, Lesley J.; Butchart, Neal; Hardiman, Steven C.; Charlton-Perez, Andrew J.; Watson, Peter (August 2012). "The Effect of Climate Change on the Variability of the Northern Hemisphere Stratospheric Polar Vortex". Journal of the Atmospheric Sciences. 69 (8): 2608–2618. Bibcode:2012JAtS...69.2608M. doi:10.1175/jas-d-12-021.1 (inactive 2 December 2024). ISSN 0022-4928. S2CID 122783377.
{{cite journal}}
: CS1 maint: DOI inactive as of December 2024 (link) - ^ Masato, Giacomo; Hoskins, Brian J.; Woollings, Tim (2013). "Winter and Summer Northern Hemisphere Blocking in CMIP5 Models". Journal of Climate. 26 (18): 7044–7059. Bibcode:2013JCli...26.7044M. doi:10.1175/JCLI-D-12-00466.1 (inactive 2 December 2024).
{{cite journal}}
: CS1 maint: DOI inactive as of December 2024 (link) - ^ Liu, Jiping; Curry, Judith A.; Wang, Huijun; Song, Mirong; Horton, Radley M. (27 February 2012). "Impact of declining Arctic sea ice on winter snowfall". PNAS. 109 (11): 4074–4079. Bibcode:2012PNAS..109.4074L. doi:10.1073/pnas.1114910109. PMC 3306672. PMID 22371563.
- ^ Qiuhong Tang; Xuejun Zhang; Francis, J. A. (December 2013). "Extreme summer weather in northern mid-latitudes linked to a vanishing cryosphere". Nature Climate Change. 4 (1): 45–50. Bibcode:2014NatCC...4...45T. doi:10.1038/nclimate2065.
- ^ Screen, J A (November 2013). "Influence of Arctic sea ice on European summer precipitation". Environmental Research Letters. 8 (4): 044015. Bibcode:2013ERL.....8d4015S. doi:10.1088/1748-9326/8/4/044015. hdl:10871/14835.
- ^ Friedlander, Blaine (4 March 2013). "Arctic ice loss amplified Superstorm Sandy violence". Cornell Chronicle. Archived from the original on 11 June 2015. Retrieved 7 January 2014.
- ^ Walsh, Bryan (6 January 2014). "Polar Vortex: Climate Change Might Just Be Driving the Historic Cold Snap". Time. Archived from the original on 11 January 2018. Retrieved 7 January 2014.
- ^ Spotts, Pete (6 January 2014). "How frigid 'polar vortex' could be result of global warming (+video)". The Christian Science Monitor. Archived from the original on 9 July 2017. Retrieved 8 January 2014.
- ^ Jennifer Francis; Natasa Skific (1 June 2015). "Evidence linking rapid Arctic warming to mid-latitude weather patterns". Philosophical Transactions. 373 (2045): 20140170. Bibcode:2015RSPTA.37340170F. doi:10.1098/rsta.2014.0170. PMC 4455715. PMID 26032322.
- ^ Mann, Michael E.; Rahmstorf, Stefan (27 March 2017). "Influence of Anthropogenic Climate Change on Planetary Wave Resonance and Extreme Weather Events". Scientific Reports. 7: 45242. Bibcode:2017NatSR...745242M. doi:10.1038/srep45242. PMC 5366916. PMID 28345645.
- ^ "Extreme global weather is 'the face of climate change' says leading scientist". The Guardian. 2018. Archived from the original on 13 April 2019. Retrieved 8 October 2022.
- ^ Francis J; Vavrus S; Cohen J. (2017). "Amplified Arctic warming and mid latitude weather: new perspectives on emerging connections" (PDF). Wiley Interdisciplinary Reviews: Climate Change. 8 (5). 2017 Wiley Periodicals,Inc: e474. Bibcode:2017WIRCC...8E.474F. doi:10.1002/wcc.474. Archived (PDF) from the original on 21 March 2023. Retrieved 8 October 2022.
- ^ Fischetti, Mark (2017). "The Arctic Is Getting Crazy". Scientific American. Archived from the original on 22 April 2022. Retrieved 8 October 2022.
- ^ Kretschmer, Marlene; Coumou, Dim; Agel, Laurie; Barlow, Mathew; Tziperman, Eli; Cohen, Judah (January 2018). "More-Persistent Weak Stratospheric Polar Vortex States Linked to Cold Extremes" (PDF). Bulletin of the American Meteorological Society. 99 (1): 49–60. Bibcode:2018BAMS...99...49K. doi:10.1175/bams-d-16-0259.1 (inactive 2 December 2024). ISSN 0003-0007. S2CID 51847061. Archived (PDF) from the original on 9 October 2022. Retrieved 8 October 2022.
{{cite journal}}
: CS1 maint: DOI inactive as of December 2024 (link) - ^ Coumou, D.; Di Capua, G.; Vavrus, S.; Wang, L.; Wang, S. (2018-08-20). "The influence of Arctic amplification on mid-latitude summer circulation". Nature Communications. 9 (1): 2959. Bibcode:2018NatCo...9.2959C. doi:10.1038/s41467-018-05256-8. ISSN 2041-1723. PMC 6102303. PMID 30127423.
- ^ Kim, Jin-Soo; Kug, Jong-Seong; Jeong, Su-Jong; Huntzinger, Deborah N.; Michalak, Anna M.; Schwalm, Christopher R.; Wei, Yaxing; Schaefer, Kevin (26 October 2021). "Reduced North American terrestrial primary productivity linked to anomalous Arctic warming". Nature Geoscience. 10 (8): 572–576. doi:10.1038/ngeo2986. OSTI 1394479. Archived from the original on 28 November 2022. Retrieved 15 October 2022.
- ^ "Climate change: Arctic warming linked to colder winters". BBC News. 2 September 2021. Archived from the original on 20 October 2021. Retrieved 20 October 2021.
- ^ Cohen, Judah; Agel, Laurie; Barlow, Mathew; Garfinkel, Chaim I.; White, Ian (3 September 2021). "Linking Arctic variability and change with extreme winter weather in the United States". Science. 373 (6559): 1116–1121. Bibcode:2021Sci...373.1116C. doi:10.1126/science.abi9167. PMID 34516838. S2CID 237402139. Archived from the original on 16 April 2023. Retrieved 8 October 2022.
- ^ Zou, Yofei; Rasch, Philip J.; Wang, Hailong; Xie, Zuowei; Zhang, Rudong (26 October 2021). "Increasing large wildfires over the western United States linked to diminishing sea ice in the Arctic". Nature Communications. 12 (1): 6048. Bibcode:2021NatCo..12.6048Z. doi:10.1038/s41467-021-26232-9. PMC 8548308. PMID 34702824. S2CID 233618492.
- ^ Weng, H. (2012). "Impacts of multi-scale solar activity on climate. Part I: Atmospheric circulation patterns and climate extremes". Advances in Atmospheric Sciences. 29 (4): 867–886. Bibcode:2012AdAtS..29..867W. doi:10.1007/s00376-012-1238-1. S2CID 123066849.
- ^ James E. Overland (December 8, 2013). "Atmospheric science: Long-range linkage". Nature Climate Change. 4 (1): 11–12. Bibcode:2014NatCC...4...11O. doi:10.1038/nclimate2079.
- ^ Seviour, William J.M. (14 April 2017). "Weakening and shift of the Arctic stratospheric polar vortex: Internal variability or forced response?". Geophysical Research Letters. 44 (7): 3365–3373. Bibcode:2017GeoRL..44.3365S. doi:10.1002/2017GL073071. hdl:1983/caf74781-222b-4735-b171-8842cead4086. S2CID 131938684.
- ^ Screen, James A. (15 June 2014). "Arctic amplification decreases temperature variance in northern mid- to high-latitudes". Nature Climate Change. 4 (7): 577–582. Bibcode:2014NatCC...4..577S. doi:10.1038/nclimate2268. hdl:10871/15095. Archived from the original on 23 February 2022. Retrieved 8 October 2022.
- ^ van Oldenborgh, Geert Jan; Mitchell-Larson, Eli; Vecchi, Gabriel A.; de Vries, Hylke; Vautar, Robert; Otto, Friederike (22 November 2019). "Cold waves are getting milder in the northern midlatitudes". Environmental Research Letters. 14 (11): 114004. Bibcode:2019ERL....14k4004V. doi:10.1088/1748-9326/ab4867. S2CID 204420462.
- ^ Blackport, Russell; Screen, James A.; van der Wiel, Karin; Bintanja, Richard (September 2019). "Minimal influence of reduced Arctic sea ice on coincident cold winters in mid-latitudes". Nature Climate Change. 9 (9): 697–704. Bibcode:2019NatCC...9..697B. doi:10.1038/s41558-019-0551-4. hdl:10871/39784. S2CID 199542188.
- ^ Blackport, Russell; Screen, James A. (February 2020). "Insignificant effect of Arctic amplification on the amplitude of midlatitude atmospheric waves". Science Advances. 6 (8): eaay2880. Bibcode:2020SciA....6.2880B. doi:10.1126/sciadv.aay2880. PMC 7030927. PMID 32128402.
- ^ Streffing, Jan; Semmler, Tido; Zampieri, Lorenzo; Jung, Thomas (24 September 2021). "Response of Northern Hemisphere Weather and Climate to Arctic Sea Ice Decline: Resolution Independence in Polar Amplification Model Intercomparison Project (PAMIP) Simulations". Journal of Climate. 34 (20): 8445–8457. Bibcode:2021JCli...34.8445S. doi:10.1175/JCLI-D-19-1005.1 (inactive 2 December 2024). S2CID 239631549.
{{cite journal}}
: CS1 maint: DOI inactive as of December 2024 (link) - ^ Paul Voosen (2021-05-12). "Landmark study casts doubt on controversial theory linking melting Arctic to severe winter weather". Science Magazine. Archived from the original on 9 March 2023. Retrieved 7 October 2022.
- ^ Smith, D.M.; Eade, R.; Andrews, M.B.; et al. (7 February 2022). "Robust but weak winter atmospheric circulation response to future Arctic sea ice loss". Nature Communications. 13 (1): 727. Bibcode:2022NatCo..13..727S. doi:10.1038/s41467-022-28283-y. PMC 8821642. PMID 35132058. S2CID 246637132.
- ^ Martin, Jonathan E. (14 April 2021). "Recent Trends in the Waviness of the Northern Hemisphere Wintertime Polar and Subtropical Jets". Journal of Geophysical Research: Atmospheres. 126 (9). Bibcode:2021JGRD..12633668M. doi:10.1029/2020JD033668. S2CID 222246122. Archived from the original on 15 October 2022. Retrieved 8 October 2022.
- ^ Tenenbaum, Joel; Williams, Paul D.; Turp, Debi; Buchanan, Piers; Coulson, Robert; Gill, Philip G.; Lunnon, Robert W.; Oztunali, Marguerite G.; Rankin, John; Rukhovets, Leonid (July 2022). "Aircraft observations and reanalysis depictions of trends in the North Atlantic winter jet stream wind speeds and turbulence". Quarterly Journal of the Royal Meteorological Society. 148 (747): 2927–2941. Bibcode:2022QJRMS.148.2927T. doi:10.1002/qj.4342. ISSN 0035-9009. S2CID 250029057.
- ^ Osman, Matthew B.; Coats, Sloan; Das, Sarah B.; McConnell, Joseph R.; Chellman, Nathan (13 September 2021). "North Atlantic jet stream projections in the context of the past 1,250 years". PNAS. 118 (38). Bibcode:2021PNAS..11804105O. doi:10.1073/pnas.2104105118. PMC 8463874. PMID 34518222.
External links
- Turton, Steve (3 June 2021). "Why is the Arctic warming faster than other parts of the world? Scientists explain". WEForum.org. World Economic Forum. Archived from the original on 3 June 2021.